Detecting Dark Matter
A brief overview of the experimental search for dark matter (XENON, CDMS, PICASSO, COUPP).
Want to get notified about new posts? Join the mailing list and follow on X/Twitter.
Although the existence of dark matter is supported by a variety of strong observational evidence, very little is known about its nature. Several plausible dark matter candidates have been proposed, but their weak interactivity with normal matter makes them extremely difficult to detect.
For this reason, the only known way to directly detect dark matter is to detect nuclei scattered by collisions and rule out all sources of nuclear recoils from known particles. Experiments such as XENON [19], CDMS [20], PICASSO [21], and COUPP [22] have attempted to detect and discriminate nuclear collisions using two-phase noble gas chambers, cryogenic detectors, liquid Freon droplet detectors, and bubble chambers.
The first step to identifying dark matter candidates was to determine whether the collisions are caused by baryonic matter or nonbaryonic dark matter. Baryonic matter, or “normal” matter, includes objects composed of quarks and leptons, the building blocks of atoms. The most likely baryonic candidates for dark matter, MACHOs (short for Massive Compact Halo Objects), can be faint red stars, brown dwarfs, or other Jupiter-size objects that don’t emit large amounts of light [23]. They can be detected by gravitational microlensing, which occurs when a MACHO gravitational lens passes in front of a luminous object. The MACHO bends the background light, brightening distant stars for a short time [24]. Although MACHOs have been detected, there are not nearly enough to account for the mass of dark matter [23].
Unlike baryonic matter, nonbaryonic matter consists of particles we have yet to discover. In the past, the most considered nonbaryonic dark matter candidate was the neutrino. Neutrinos exhibit many of the characteristics displayed by dark matter—they are neutral and incapable of emitting electromagnetic radiation, and they only interact through the gravitational and weak forces [23]. However, being “hot,” they move too quickly to clump into dark matter halos. This led to the proposal of WIMPs, or weakly interacting massive particles, which exhibit the aforementioned characteristics but are “cold” and move slowly enough to clump [23]. The most studied WIMP candidate is the neutralino, which is predicted by supersymmetry, a theoretical extension of the Standard Model that hypothesizes a heavier counterpart for every known particle. Neutralinos are plausible candidates for dark matter because they are heavy and neutral, emit no electromagnetic radiation, do not collide often, and are stable since they are the lightest supersymmetric particles [25].
Numerous dark matter searches attempt to directly detect WIMPs, and they all follow the same principle of creating a particle-scattering detection system and ruling out collisions due to known particles to discern dark matter. This is done by minimizing background radiation and utilizing a variety of methods to discriminate particle collisions. For example, cryogenic detectors use thermistors to measure temperature changes which may be attributed to collisions between WIMPs and target nuclei, scintillation detectors analyze scintillation light that is emitted when electrons excited by collisions relax to lower energy states [26], ionization detectors detect charged ions formed by particle collisions, and bubble chamber detectors detect bubbles that form when a particle hits a nucleus of a superheated liquid and the recoiling nucleus loses kinetic energy as heat to the surrounding liquid [27]. Because so little is known about dark matter and the sensitivity required to detect it [28], it is essential that dark matter detectors are as sensitive as possible.
XENON
An excellent example of a dark matter search that pairs scintillation and ionization detecting techniques is XENON, which uses two chambers containing liquid xenon and gaseous xenon as a means to detect particle collisions. Such collisions can excite electrons in the xenon atoms, and the electrons emit scintillation light when they return to their original states [26]. Photomultipliers placed above the gas and below the liquid [30] then amplify the light to detectable levels [29].
Sometimes, if the collision is energetic enough, electrons are freed from their nuclear orbits. Although such an event does not emit light, an applied voltage moves the unbound electrons toward the xenon gas [29] where their charges can be detected. The charge-to-light ratio of an event is then used to determine whether it is an electronic recoil (from electrons or photons) or a nuclear recoil.
To prevent radiation contamination, the xenon is continuously recirculated and purified [31]. Data from XENON100’s 225 day collection has placed strict limits on dark matter characteristics [30], and the XENON collaboration plans to launch an improved detector, XENON1T, in 2015 [32].
CDMS
In the Soudan Underground Laboratory in Minnesota [20], CDMS (the Cryogenic Dark Matter Search) attempts to detect WIMPs by detecting phonon and ionization signals within a detector crystal. When a particle collides with a nucleus in the detector, vibrations known as phonons propagate through the crystal and reach aluminum collector fins. The energy from the phonons is transferred to the aluminum electrons, which travel to and change the resistance of tungsten strips. This change in resistance can be amplified to make a detectable pulse.
In addition to creating phonons, particle collisions in the detector can also result in ionization and free electrons. An applied voltage moves the electrons toward the crystal surface, where they are detected by a charge amplifier [33]. Cosmic radiation is suppressed by CDMS’s underground location [20], and recoils are discriminated by the amount of ionization they produce and the shapes of the resulting phonon pulses [33].
CDMS has not been able to detect dark matter yet, but it has succeeded in setting more constraints on dark matter characteristics. Its most current experiment is SuperCDMS, which will eventually be moved 2 kilometers underground in SNOLAB to improve cosmic background suppression [20].
PICASSO
Launched in 2000 [25], PICASSO (Project in CAnada to Search for Supersymmetric Objects) searches for dark matter particles using millions of 50-100 micrometer liquid Freon droplets. These droplets are superheated so that the kinetic energy from particle collisions initiates bubble formation in the droplets. When the bubbles become large enough, they cause the droplets to pop.
Although PICASSO’s underground location and water cube barrier block much of the cosmic and neutron radiation, there is still some background that has to be accounted for. One method of discrimination involves analyzing the acoustic emissions when the droplets burst. Piezoelectric sensors are used to detect the emissions [28], and the type of particle involved in each collision can be determined from the amplitude of the resulting acoustic signal [34].
Another technique used to discriminate background radiation involves adjusting the temperature and pressure of the Freon droplets to adjust the bubble nucleation threshold, or the energy and length the energy needs to be released over to form a bubble [28]. Alpha particles are detectable above $15^\circ$ C, neutralinos above $30^\circ$ C, and gamma rays and other minimum ionizing particles above $50^\circ$ C. To take advantage of this, the detector operates at different temperatures ranging from $20-47^\circ$ C.
Although PICASSO has not found evidence for any dark matter collisions, it has gathered enough information to help narrow down the characteristics of these mysterious dark matter particles [28].
COUPP
Like PICASSO, the COUPP experiment (Chicagoland Observatory for Underground Particle Physics) also employs explosive phase transition in superheated liquid. However, the COUPP experiment takes a slightly different approach. Rather than Freon droplets, COUPP uses superheated liquid CF3I as a target for the collisions. The CF3I is located in a fused silica bell jar in a pressure vessel surrounded by propylene glycol for pressure balancing. The kinetic energy transferred to C, F, or I nuclei in the collisions causes bubbles to form in the CF3I [35].
The testing period begins with decompression for 5 seconds, which allows the CF3 I to superheat. Then, the pressure is stabilized for 30 seconds before the vessel enters a 500 second waiting period. If a bubble forms during this interval, the vessel is compressed for 30 seconds to keep it from expanding uncontrollably. If no bubbles are detected, compression occurs for 30 seconds at the end of the 500 second waiting period, and the cycle repeats [27].
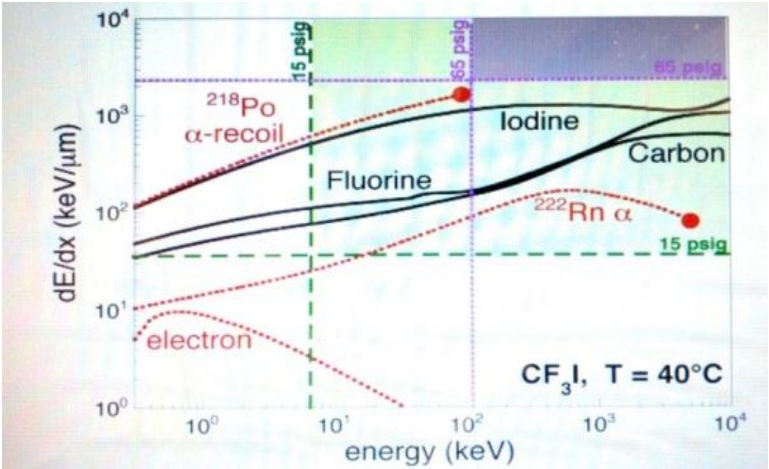
Figure 5: Dependence of bubble formation on total energy and stopping power [36].
In order to identify the bubbles caused by WIMP collisions, background has to be limited and recoils of known particles have to be identified. Background limitation is achieved by adjusting the temperature and pressure of the vessel [27] so only particles with large stopping power (energy deposition per unit length) (see Figure 5) are capable of fulfilling the bubble nucleation threshold and triggering bubbles [37]. COUPP’s location 6800 feet underground (at SNOLAB near Sudbury, Ontario, Canada) provides a measurement area mostly free of cosmic ray background, but alpha emissions due to the radioactivity of some of the materials surrounding the CF3 I and in the rock walls still contribute to background radiation [27]. To discriminate the events, multiple characteristics of the bubbles are analyzed such as bubble location, the number of bubbles, and the acoustic emissions from the bubbles.
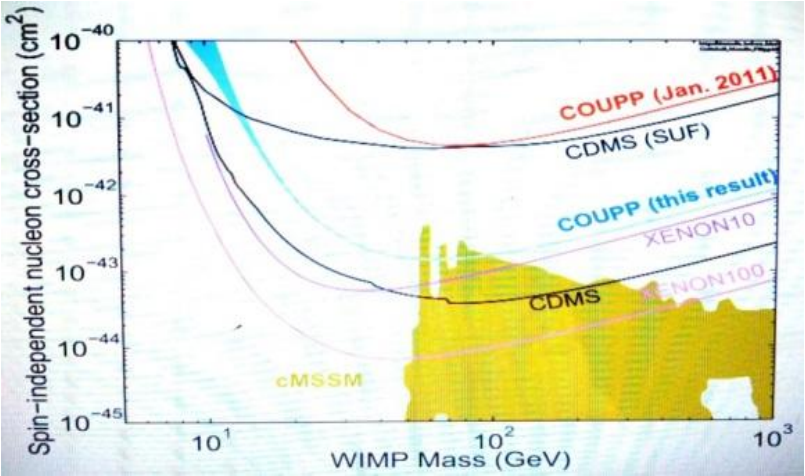
Figure 6: WIMP limits from recent dark matter searches [38].
Like XENON100, CDMS, and PICASSO, COUPP has not found evidence for dark matter collisions but has succeeded in setting new WIMP limits (see Figure 6) and plans to launch new and improved experiments [36].
References
[19] XENON Collaboration. The XENON Dark Matter Project. Columbia University, 17 2011. Web. Web. 26 Oct. 2012. (link)
[20] Super Cryogenic Dark Matter Search. University of California, Berkeley, n. d. Web. Web. 20 Oct. 2012. (link)
[21] The Picasso Experiment. N.p., n. d. Web. Web. 13 Oct. 2012. (link)
[22] COUPP Experiment - E961. COUPP Collaboration, 8 Dec. 2006. Web. 26 Oct. 2012. (link)
[23] Bennett, Jeffery, et al. The Cosmic Perspective. 3rd ed. San Francisco: Addison Wesley, 2004. 679-696. Print.
[24] Massey, Richard, Thomas Kitching, et al. “The dark matter of gravitational lensing.” Rep. Prog. Phys. 73. (2010): n.pag. ArXiv. Database. 28 Sept. 2012.
[25] Cline, David B. “The Search for Dark Matter.” Scientific American. 52-59. March 2003. Print. 20 Sept. 2012.
[26] Tro, Nivaldo J. Chemistry: a Molecular Approach. 2nd ed. Upper Saddle River: Pearson Education, Inc., 2011. 289-90. Print.
[27] Behnke, E., J. Behnke, et al. “First dark matter search results from a 4-kg CF3I bubble chamber operated in a deep underground site.” Phys. Rev. D. 86. (2012): 933-936. ArXiv. Database. 4 Oct. 2012.
[28] “Experiment.” The Picasso Experiment. N.p., n.d. Web. 13 Oct 2012. (link)
[29] XENON100 Collaboration. “The XENON100 Dark Matter Experiment.” Astropart. Phys. 35. (2012): 573-590. ArXiv. Database. 13 Oct 2012.
[30] XENON100 Collaboration. “Dark Matter Results from 100 Live Days of XENON100 Data.” Phys. Rev. Lett. 107. (2011): 573-590. ArXiv. Database. 13 Oct 2012.
[31] Giboni, K. L., E. Aprile, et al. “Xenon RecirculationPurification with a Heat Exchanger.” JINST 6. (2011): 573-590. ArXiv. Database. 13 Oct 2012.
[32] Aprile, Elena. “The XENON1T: Experiment at LNGS.” DM2012. 24 Feb. 2012. (link)
[33] CDMS II Detectors. University of California, Berkeley, 28 Apr. 2007. Web. 22 Oct. 2012. (link)
[34] Davour, A. “The PICASSO Dark Matter Search Project.” Proc. of Identification of Dark Matter 2008 (2010): n.pag. The Picasso Experiment. 28 Sept. 2012.
[35] Conner, Austin. Design, Construction, and Commissioning of a COUPP Pressure Vessel Simulator. (2011): 1-11. Web. 20 Sept. 2012.
[36] Collar, Juan, Eric C. Dahl, et al. “COUPP *-500: A Proposal for a Ton Scale Bubble Chamber for Dark Matter Detection.” (2010): 1,3,30. Coupp Experiment-E961. Web. 26 Oct 2012.
[37] Behnke, E., J.I. Collar, et al. “Improved SpinDependent WIMP Limits from a Bubble Chamber.” Science. 319. (2008): 933-936. ArXiv. Database. 20 Sept 2012.
[38] Behnke, E., J. Behnke, et al. “First dark matter search results from a 4-kg CF3I bubble chamber operated in a deep underground site.” Phys. Rev. D. 86. (2012): 933-936. ArXiv. Database. 4 Oct. 2012.
Want to get notified about new posts? Join the mailing list and follow on X/Twitter.